Ever since the discovery and cloning of the basic cytokines that promoted differentiation of haematopoietic stem cells towards erythroid or megakaryocyte lineages, we have been aware of the potential to produce red cells and platelets in vitro for transfusion to humans. Here, we describe the next steps towards this goal.
The drivers and the discoveries
Provision of safe blood
Every year, the UK requires thousands of voluntary blood donations to supply nearly 2 million units of red cells and 280,000 units of platelets to patients in need.
The recent pandemic and resultant staff shortages have illustrated that supply lines remain precarious, even in a developed country like the UK. In other parts of the developed world, major weather phenomena have also caused blood product shortages in the last few years.
The use of allogeneic products will always be associated with adverse events such as red cell alloimmunisation and the risk of transmission of infectious agents. The UK is only just now grasping the consequences of HIV and hepatitis C transmission through the blood supply line.
Of course, there is an ongoing need to adapt and change the screening and testing of blood, given the continuing emergence of viruses transmissible through blood (hepatitis E, Zika) or indeed entirely novel infections (such as variant Creutzfeldt–Jakob disease). In developing countries with endemic infections such as HIV or hepatitis B, the provision of safe blood is even today a major hurdle for the delivery of healthcare.
Genome editing has allowed the production of non-immunogenic 'universal' blood cells or blood cells negative for antigens...
Producing red blood cells in vitro
In the 1990s and 2000s, protocols were established to produce small quantities of red cells from primary stem cells; this allowed the dream of supplementing donated red cells with in-vitro-derived red cells to finally take shape. With the emergence of pluripotent stem cell technologies (first embryonic stem cells, then induced pluripotent stem cells) allowing the expansion and maintenance of stem cell lines, we took a further step towards large-scale production – or so we thought.
Erythroblasts derived from pluripotent stem cells are fundamentally different from those derived from either cord blood- or adult peripheral-blood CD34+ haematopoietic progenitors. They contain predominantly embryonic globins that do not transport oxygen effectively to peripheral tissues and, more crucially, do not enucleate. These limitations represent major hurdles towards the clinical translation of red cell production in vitro.1
Genomics
As these difficulties arose, other technologies appeared to boost the production of blood cells in vitro for human use, in particular the emergence of affordable, efficient and accurate genome editing. It has been clear for a few years that the production of blood cells in vitro will not supplant donor-based supply; the overwhelming safety, efficacy and relatively cheap costs of donor-sourced blood products make this virtually impossible. Instead, ideas are evolving for advanced blood cell therapies that fill an unmet need.
Genome editing has allowed for the emergence of novel concepts: the production of non-immunogenic ‘universal’ blood cells or blood cells negative for antigens that otherwise make transfusion of multi-alloimmunised patients extremely difficult (for example, for patients with complex and multiple alloantibodies or anti-U).2 In the case of platelets, it may be possible to produce HLA null platelets, platelets with enhanced haemostatic properties, or even platelets that are not damaged in cold storage.
In vitro red cells for iron overload
In the world of red cell transfusion, one particular aspect of clinical care that would benefit from the use of red cells made in vitro is the challenge of iron overload. Patients who require chronic transfusion support have limited capabilities to excrete the iron transfused with each red cell unit. This can lead to end organ damage, including heart failure, liver disease and endocrine disorders. For patients who have undergone curative therapies for haematological malignancies or aplastic anaemia, iron overload can be addressed by venesection upon bone marrow recovery. The challenge remains for people with inherited red cell disorders, particularly those with thalassaemia major or sickle cell disease. For these patients, where transfusion every 2–3 weeks is a requirement to stay alive, iron chelation therapy is an absolute necessity to prevent end organ damage early in adult life. Moreover, iron chelation therapy is not easy to implement. Oral agents, once thought to provide a solution, are unfortunately inefficient compared to subcutaneous desferrioxamine.3,4 This treatment is poorly tolerated and a lack of compliance, particularly in teenagers and young adults, is a major hurdle to successful implementation.
A pack of red cells derived from a donation will contain a mixture of red cells, some of which are newly emerged from the bone marrow and some of which have been circulating for 4 months and are very near the end of their life cycle. This is why, although red cells have a life span of 120 days, patients need to be transfused every 2–3 weeks. Hypothetically, in-vitro-derived red cells would have a synchronised birth in the culture system and would only be a few days old when transfused. This, in turn, would lead to much longer survival in circulation, allowing a much larger gap between transfusions for the patient and thereby slowing down iron accumulation.
The clinical trial and the challenges
RESTORE trial background
This particular challenge is being addressed by the RESTORE trial, jointly funded by the National Institute for Health and Care Research (NIHR) and NHS Blood and Transplant (NHSBT). The trial is based on a culture protocol published 10 years ago by Professor Anstee in Bristol,5 demonstrating that erythroblasts cultured in vitro from peripheral blood CD34+ progenitors are able to efficiently enucleate, producing reticulocytes that are phenotypically similar to donor-derived red cells.6
RESTORE is a first-in-human crossover study investigating whether red cells derived in vitro survive better than donor-derived red cells in the circulation. For the purpose of the trial, a mini-dose of red cells labelled with Cr51 (a radionuclide that efficiently and almost irreversibly binds to red cells, used for decades in red cell survival studies) is transfused to a healthy volunteer (see Figure 1). The survival curve of cells is then assessed by regular sampling over 120 days.
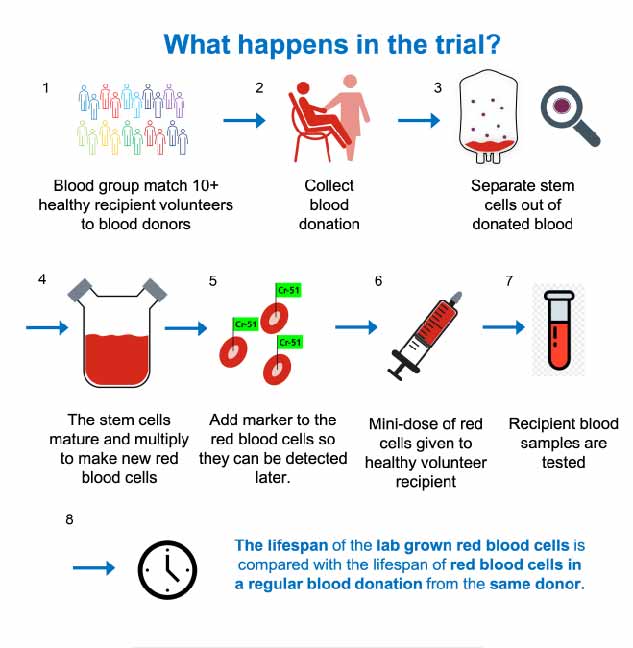
Each volunteer is randomised to initially receive donated red cells or manufactured red cells and then receives the other cell type 6 months later. The donors are recruited from the Cambridge blood
donor centre and are giving 2 donations: 1 that will produce standard red cells, the other providing the haematopoietic progenitor cells from which the manufactured red cells are produced. Each donor–recipient pair is matched for the most clinically relevant antigens (ABO, full Rh profile, Kell, Duffy, Kid and Ss blood groups), made possible by the recruitment of volunteers from a fully phenotyped/genotyped cohort of 10,000 people in the Cambridgeshire area (thanks to Cambridge BioResource).
...we have now reached the stage where manufactured red cells are being transfused to humans; a triumph of science, this is due to the dedication and skills from a very large team...
Trial funding and staffing
Although the theory can be explained in one paragraph, the practicalities of setting up and seeing through such a trial are not to be underestimated. First, as ever, is the question of funding. Even at low scale and for first-in-human studies such as RESTORE, cellular therapy trials command a 7-figure bill, which requires cooperation of funding bodies. In the case of RESTORE, support came from a Blood Transfusion Research Unit led by Professor Ash Toye (University of Cambridge) funded by the NIHR and by additional funding from NHSBT.
Second, the breadth of expertise requires a huge team of people. This includes medical teams comprising research nurses and medics; clinical trial teams (trial and data manager, statisticians, in this case supported by the Clinical Trial Unit of NHSBT); quality control staff and qualified persons; good manufacturing practice (GMP) facility-trained staff (NHSBT, Bristol); and staff from the nuclear medicine department (Guy's Hospital for the labelling and Cambridge United Hospitals for data) and clinical units (blood donor centre in Cambridge, early phase clinical research unit, Cambridge United Hospitals). External governance through a trial steering committee and programme board is also essential, not only as a sounding board for the executive team overseeing the trial, but also providing the governance that is required.
...the RESTORE team has paved the way for future trials with cellular therapies and advanced blood products...
Imponderables and curve balls
And then there are the elements that we all tend to ignore: the imponderables and the need for resilience and creativity to reach the finishing line. I will mention here just a few issues that RESTORE has encountered. These are words of warning to those who are looking to deliver cell therapy trials, but also to the organisations supporting them and their funders: contingency plans and not holding anyone to the impossible are conditions sine qua non!
Supply lines will be a continuing worry for any trial. Transferring R&D culture protocol to GMP will often lead to single-supplier issues. Reagents suitable for clinical-grade cell production need to be validated for the investigational medicinal product dossier submission to the regulator. However, suppliers can discontinue products or reformulate them, leading to the need to stock up (and assess stability over time) or revalidate reagents (which is time-consuming and costly).
Training and retaining highly qualified staff for long periods of time is in itself a challenge. Training staff in GMP facilities takes about 6 months and, as more cellular therapies continue to emerge, the demand for such staff in both academia and industry is building.
Despite these challenges, we have now reached the stage where manufactured red cells are being transfused to humans; a triumph of science.
Then there are the curve balls. Red cells are anucleate and, therefore, the final product in the trial is not an Advanced Therapy Medicinal Product (e.g. a cellular therapy) but an Investigational Medicinal Product (e.g. a drug), which requires a different approach to regulatory approval. There are also the imponderables we could never have suspected or planned for. The COVID-19 pandemic put everything on hold for 18 months. It was challenging adapting testing and recruitment in an ever-changing landscape to keep participants and staff safe. An outbreak of Lhassa fever in the Cambridgeshire area led to a temporary lack of availability of intensive care beds (a legal requirement for any first-in-human trial).
The future
Despite these challenges, we have now reached the stage where manufactured red cells are being transfused to humans; a triumph of science. This is due to the dedication and skills of a very large team and commitment by funders and organisations across the breadth of the UK. The potential benefits of the trial reach far beyond any results it may yield. The learning curve experienced by the RESTORE team has paved the way for future trials with cellular therapies and advanced blood products, bringing us one step closer to treating patients in need with these transformative therapies.